Understanding Battery Types, Components and the Role of Battery Material Testing in Development and Manufacture
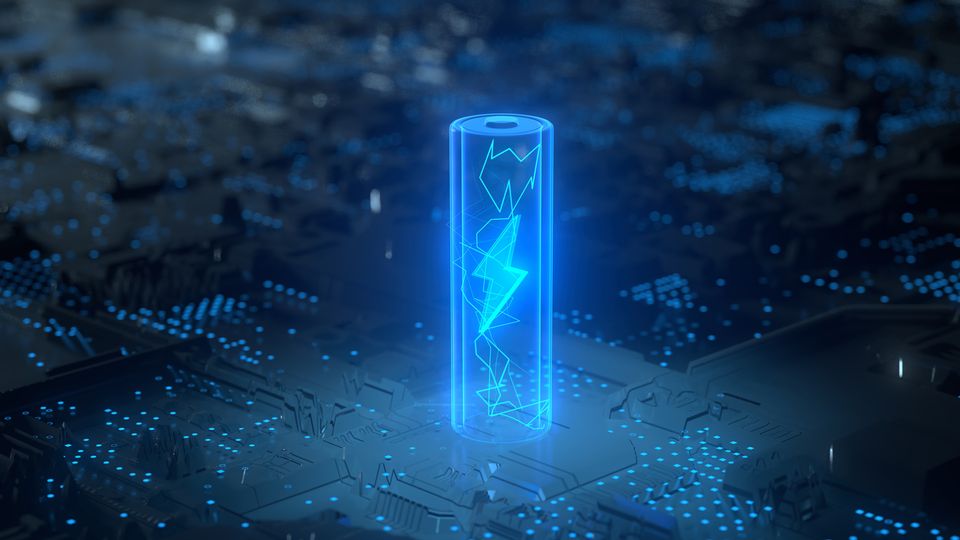
Complete the form below to unlock access to ALL audio articles.
Any device that can transform its chemical energy into electrical energy through reduction-oxidation (redox) reactions involving its active materials, commonly known as electrodes, is pedagogically now referred to as a battery.1 Essentially, a battery contains one or many identical cells that each stores electrical power as chemical energy in two electrodes that are separated by an electrolyte.2
Who invented the battery?
What are the main different types of batteries?
- Primary batteries
- Alkaline battery
- Lithium metal battery
- Secondary batteries
- Nickel metal hydride battery
- Lead acid battery
- Lithium ion battery
- Solid state battery
What are batteries made of and what are the main battery components?
- Battery separator
- Battery electrolyte
- Anode
- Cathode
- Current collectors
How are batteries made and why might you test a battery material?
- Battery material impurity
- Battery safety
- Thermal runaway
- Battery degradation
- Cost reduction
Analytical testing in battery manufacturing
- Raw materials analysis
- Battery slurry analysis
- Electrode analysis
- Electrolyte analysis
- Battery performance testing
By immersing two different metals or metal compounds (electrodes) into an ion-conducting system (electrolyte), electrons tend to move from one electrode to the other, utilizing the basic electrochemical property of the electrodes. When a load is connected, electrons start flowing from one electrode to the other, generating electricity. This is called discharging. During the charging or recharging process, an opposite potential is applied to the electrodes, causing electrons to return to their original positions. Usually, an ion-porous separator is placed in the electrolyte between the two electrodes to prevent short circuiting. Figure 1 shows a schematic of the first lithium ion (Li–ion) rechargeable battery.3
Figure 1: Schematic illustration of the first Li-ion battery. Credit: The Author, adapted from Goodenough et al.3
In this article, we will consider the main types of batteries, battery components and materials and the reasons for and ways in which battery materials are tested.
Who invented the battery?
Batteries are perhaps the most prevalent and oldest forms of energy storage technology in human history.4 Nonetheless, it was not until 1749 that the term "battery" was coined by Benjamin Franklin to describe several capacitors (known as Leyden jars, after the town in which it was discovered), connected in series. The term "battery" was presumably chosen based on the analogy to existing terminology used to describe a grouping of similar equipment operating collectively, like a battery of artillery guns. Interestingly, in present times, unless explicitly specified otherwise, the term "battery" universally refers to electrochemical cells used for generating electrical energy, and even a single cell is now referred to as a battery.
Along the timeline of technological development, several notable advancements have played a significant role in shaping and evolving modern batteries. It was speculated that the inhabitants of the Parthian civilization in the 1700s electroplated gold onto silver using jars comprised of an iron rod within a copper cylinder, an assemblage which is known as the “Bagdad Battery”. However, Alexander Volta is considered the real discoverer of batteries.1 He made and introduced the first successful demonstration of a modern battery in 1800, commonly referred to as the Voltaic pile. Other developments include the Daniel cell in 1836 and the first rechargeable battery, the lead–acid battery, in 1854. Lithium-based batteries were the last to emerge in the progression of battery technology, only introduced in the 1970s. Figure 2 illustrates the timeline of introduction of the common types of batteries.
Figure 2: The historical timeline of the introduction of some common batteries. Credit: The Author.
What are the main different types of batteries?
There are two main types of batteries. These are primary batteries and secondary batteries. Table 1 provides an overview of the principal commercial battery chemistries, together with their class (primary/secondary) and examples of typical application areas. Let’s consider the more common types in more detail.
Primary batteries
These are also known as non-rechargeable batteries. They are designed for single use and then discarded without the possibility to be recharged. Once their energy is depleted, they need to be replaced. Primary batteries are assembled in the charged state and their capacity is limited to the amount of energy obtainable from the volume of reactants placed in them during manufacture. Figure 3 shows the process flow diagram of materials and resources through the life cycle of primary batteries.5 Notable examples of primary batteries include alkaline batteries and lithium metal batteries.
Figure 3: The process flow diagram for primary batteries. Credit: Technology Networks.
- Alkaline battery
An alkaline battery is a common type of primary battery that is widely used in various electronic devices such as flashlights, remote controls, toys and portable electronics. This type of battery typically uses zinc (Zn) as the negative electrode and manganese dioxide (MnO2) as the positive electrode, with an alkaline electrolyte, usually potassium hydroxide (KOH) in between the electrodes. Alkaline batteries offer high energy density and good performance under moderate loads with a long shelf life
- Lithium metal battery
Lithium metal batteries (not to be confused with Li–ion batteries) are a type of primary battery that uses metallic lithium (Li) as the negative electrode and a combination of different materials such as iron disulfide (FeS2) or MnO2 as the positive electrode. These batteries offer high energy density, lightweight design and excellent performance at both low and high temperatures. Lithium metal batteries offer long shelf life and reliable power. As such, they are commonly used in medical devices, watches, calculators and backup power systems.
Secondary batteries
Secondary batteries can be recharged after being discharged by reversing the flow of current through the battery. Other terms for this type of battery are rechargeable battery or accumulator. Secondary batteries are usually assembled in the discharged state and have to be charged first before they can undergo discharge in a secondary process.6 The process flow for rechargeable batteries is shown in Figure 4.5 After being manufactured, rechargeable batteries can be used by the consumer over and over again until the end of their useful life. If battery materials are recycled following disposal, the recovered metals may be used in the production of new batteries, or they may be used for another application. Secondary batteries are therefore more environmentally friendly and cost-effective in the long run compared to primary batteries. Examples of secondary batteries include nickel–metal hydride (NiMH) batteries, lead–acid batteries, Li–ion batteries and solid-state batteries.
Figure 4: The process flow diagram for secondary batteries. Credit: Technology Networks.
- Nickel metal hydride battery
The NiMH battery is a rechargeable battery that utilizes a hydrogen-absorbing alloy as the negative electrode and nickel oxide (NiO) as the positive electrode. They are commonly used in portable electronics, such as digital cameras, cordless phones and handheld gaming devices due to their relatively low cost, good energy storage capacity and the absence of toxic materials like cadmium (Cd). They, however, suffer from self-discharge and are less tolerant to overcharge.
- Lead acid battery
Lead–acid batteries are the oldest and most commonly used rechargeable battery. They consist of a lead (Pb) negative electrode and lead oxide (PbO) positive electrode submerged in a sulfuric acid (H2SO4) electrolyte. Lead–acid batteries are known for their reliability and robustness, making them suitable for applications such as automotive starting batteries, backup power systems and renewable energy storage. Although lead–acid batteries have a relatively lower energy density compared to newer battery technologies, they remain popular due to their relatively low cost and ability to deliver high currents.
- Lithium ion battery
Li–ion batteries are rechargeable batteries that use Li compounds as the active material in both positive and negative electrodes. Li–ion batteries offer high energy density and a low self-discharge rate with a lightweight design. They have a longer lifespan and higher power density compared to other rechargeable batteries. Li–ion batteries have become the standard choice for a wide range of applications including electric vehicles (EVs), mobile devices and renewable energy storage systems.
- Solid state battery
Solid-state batteries have attracted considerable attention due to their potential safety, higher energy density, faster charging capabilities, wider operating temperature ranges and cycle-life benefits. They employ a solid electrolyte instead of the liquid or gel used in other traditional batteries. Solid-state batteries are considered a promising next-generation battery technology with the potential to revolutionize various industries, including EVs and consumer electronics, by providing improved energy storage solutions with reduced environmental impact.
Table 1: Overview of common commercial battery systems together with examples of typical applications, adapted from Winter et al.7 and Smith et al.6
Type | Designation | Anode/Negative | Electrolyte | Cathode/Positive | Typical Applications |
PRIMARY | Zinc−carbon (Leclanché) | Zn | Aqueous NH4Cl or ZnCl2 | MnO2, C | Used in a wide range of small portable electronic devices; low-cost modest discharge performance; 1.5 V cell potential |
Zinc−carbon (Zinc chloride) | Zn | Aqueous ZnCl2 | MnO2 | Used in small portable electronic devices with 1.5 V cell potential | |
Alkaline−manganese | Zn | Aqueous KOH | MnO2, C | More energy and power but also more expensive; 1.5 V cell potential | |
Mercury | Zn | Aqueous NaOH or KOH | HgO, C | Previously used in hearing aids, cameras and calculators, discontinued because of Hg toxicity; 1.35 V cell potential | |
Lithium metal | Li | Li salt in organic solvent | MnOp, C | Available in a range of systems with various cathodes with voltages between 1.5 and ~ 3.6 V | |
Lithium−manganese dioxide | Li | LiCF3SO3 or LiClO4 in organic solvent | MnO2 | Operating voltage of 3 V with high specific energy and a stable discharge curve | |
Lithium−carbon monofluoride | Li | LiCF3SO3 or LiClO4 in organic solvent | CFx | Used extensively in cameras and smaller devices providing ~ 3.2 volts per cell | |
Lithium−iron sulfide | Li | LiCF3SO3 and/or LiClO4 in organic solvent | FeS2 | Deliver around 1.5 V cell voltage and operate even in extreme temperatures from as low as - 40 °C up to + 60 °C | |
Lithium−iodine | Li | LiI in organic solvent | I2 | Commonly used in medical devices, such as pacemakers and implantable medical devices. Around 2.7 V cell potential | |
Lithium−silver−vanadium oxide | Li | LiAsF in organic solvent | Ag2V4O11 | Typically used in medical devices, electronics and military equipment with around 3 V cell potential | |
Lithium−sulfur dioxide | Li | SO2−LiBr in organic solvent | SO2 (C) | Used in applications that require high energy density, such as military and aerospace applications | |
Lithium−thionyl chloride | Li | SOCl2−LiAlCl4 | SOCl2 (C) | Used in applications that require long-term energy storage, such as utility metering, remote monitoring and security systems | |
Lithium−iron sulfide (thermal) | Li | Molten salt mixture LiCl−LiBr−LiF | FeS2 | Used in high-temperature applications, such as thermal batteries for military and aerospace systems; 1.8 to 2.2 V cell potential | |
Magnesium−silver chloride | Mg | Seawater | AgCl | Used in military and aerospace systems, due to their high energy density and long shelf life; around 1.6 V cell potential | |
Zinc−air | Zn | Aqueous KOH | Air, C | Principal niche market of hearing aids; good cell performance with nominal 1.4 V, but high self-discharge rate | |
Zinc−silver oxide | Zn | Aqueous KOH | Ag2O, C | Typical application in watches or calculators with good discharge performance. Expensive because of Ag content. Nominal 1.55 V cell potential | |
SECONDARY | Nickel cadmium | Cd | Aqueous KOH | NiO(OH) | Substantial market presence in portable devices, has a high cycle life but suffers from memory effect. Nominal 1.2 V cell potential; Cd is toxic |
Nickel metal hydride | AB5 or AB2 intermetallic compound | Aqueous KOH | NiO(OH) | Substitute for traditional NiCd cell with improvement in both electrochemical and environmental performance. Nominal 1.2 V cell potential | |
Lead−acid | Pb | Aqueous H2SO4 | PbO2 | Generally used in automotive applications, as a traction battery or as a reserve power source. It has high toxicity but is easy to recycle. Nominal 2 V cell voltage | |
Lithium ion | C, Lix | Li salt in organic solvent | Li(1−x)MnOp | High-performance cell widely used in portable electronic equipment with low environmental impact. Nominal 3.6 V cell potential | |
Lithium sulfur | Li | Li salt in organic solvent | S | Has a voltage window of 1.5 V to 3 V. Utilizes cost-effective and abundant materials, promise high energy densities (> 600 W h/kg) surpassing those of Li–ion batteries | |
Li−poly | C, Lix | Li salt in polymer gel | Li(1−x)MnOp | Proposed as substitute for Li-ion. Cheaper and safer with comparable performance and nominal 3.7 V cell voltage | |
Sodium ion | C, Nax | Na salt in organic or aqueous solvents | Na(1−x)MnOp | Lower material costs as compared to lithium-based batteries due to the abundance of sodium | |
Nickel−hydrogen | H2 (Pt) | KOH | NiOOH | Nickel–hydrogen batteries are commonly used in aerospace applications, such as satellites and space probes. Around 1.2 volts cell potential | |
Solid-state | Li | Ion conducting polymer, metal oxides, perovskite, NASICON, LISICON | Li(1−x)MnOp | Provide increased safety, decreased battery net weight and volume, higher output of energy and easier ion transfer. Suitable for applications in both mobile and small-scale sectors including transportation, aerospace, military and medical instrumentation. | |
Flow battery | C | Separate electrolyte containing redox-active species for positive and negative electrode | C | They can retain an exceptional lifetime of up to 100,000 cycles, thus corroborating their applicability in bulk energy storage systems. Cell potential is typically in the range of 1 to 2 V |
What are batteries made of and what are the main battery components?
Battery separator
A battery separator is usually a porous membrane placed between the negative and positive electrodes to keep the electrodes apart to prevent electrical short circuits.8 They should be very good electronic insulators and at the same time allow the rapid transport of ions that are needed to complete the circuit during the discharge and/or charge of the battery. The ion transport can be achieved through inherent ionic conductivity or by impregnating the separator with electrolyte. As batteries have advanced, the function of separators has become more complex and demanding. The characteristics of each available separator must be evaluated against the requirements of the battery system when selecting a separator. Key considerations that influence separator selection include the following as they must have/be: 8
- Electronic insulator properties
- Minimal electrolyte (ionic) resistance
- Mechanical and dimensional stability
- Enough physical strength to allow easy handling
- Chemical resistance to degradation by electrolyte, impurities and electrode reactants and products
- Effective in preventing migration of particles or colloidal or soluble species between the two electrodes
- Readily wetted by electrolyte
- Uniform in thickness and other properties
In many applications, a compromise in requirements for the separator must generally be made to optimize performance, safety, cost, etc. For example, if batteries are desired that have small internal resistance, they may require separators that are highly porous and thin, but the need for adequate physical strength may require that they be thick. Separators in sealed nickel–cadmium (NiCd) and NiMH batteries require high gas permeability to protect against overcharging. Li–ion cell separators should have a shutdown mechanism for enhanced safety. Alkaline battery separators need to be flexible enough to wrap around the electrodes. 8
Battery electrolyte
Alexander Volta first defined the electrolyte in 1800.9 It is an electron-insulating and ion-conductive layer, either liquid or solid, interposed between the negative and positive electrodes. Electrolytes are often thought of as liquids, such as water or other solvents, with dissolved salts, acids or alkalis. However, many batteries, including the conventional (AA/AAA/D) batteries, contain solid electrolytes that act as ionic conductors at room temperature. Although the specific characteristics of electrolytes can vary across different types of batteries, their fundamental role remains the same.
Electrolyte chemistry plays a major part in determining cell safety, cycle life and power capability. In aqueous batteries, such as lead–acid and NiMH batteries, the electrolyte is typically a water-based solution containing various salts or acids. Aqueous electrolytes offer good ionic conductivity and are generally cost-effective and are even being developed for Li–ion cells, mainly due to safety and environmental concerns.10 They, however, suffer from a narrow electrochemical stability window limited by water electrolysis.11
In 2015, the concept of “water-in-salt” electrolytes (WiSE), in contrast to typical “salt-in-water” electrolytes, was proposed showing an extended electrochemical stability window of 3.0 V.10 Currently, the state of-the-art electrolyte for Li–ion battery applications is Li salts, e.g., lithium hexafluorophosphate (LiPF6), dissolved in nonaqueous organic-based carbonate solvents, e.g., ethylene carbonate (EC) and dimethyl carbonate (DMC).12 Despite its ubiquitous use in EV applications, these organic electrolytes limit the cell safety due to their combustibility and limited cell operating temperature range of - 10 °C to 60 °C in the most optimistic scenarios.12
A recent new class of electrolytes has been developed by hybridizing aqueous with non-aqueous solvents, that inherits the non-flammability and non-toxicity characteristics from aqueous and better electrochemical stability from non-aqueous systems.13 There is, however, a strong push from the automotive industry to consider organic or inorganic solid-state electrolytes to be used by solid state batteries. These electrolytes can be ceramic- or polymer-based materials with high ionic conductivity. They enable the use of metallic lithium anodes, which can increase the energy density of the battery. The main benefit of solid-state batteries has been their increased safety, which stems from the absence of the flammable liquid electrolytes typically employed in Li–ion cells.14 Inorganic solid electrolytes could also support battery operation at low and high temperatures (for example, - 50 to 200 °C or higher) in which conventional liquid electrolytes would freeze, boil or decompose.14
Anode
The anode is the negative electrode of the battery associated with oxidative chemical reactions that release electrons into the external circuit.6 Li–ion batteries commonly use graphite, a form of carbon (C) as the anode material. Graphite has a layered structure, allowing lithium ions to be inserted into the layers during charging and extracted during discharge. However, the nature of the chemical interaction with lithium leads to low energy density.
Silicon is an alternative to graphite due to its higher theoretical capacity for lithium ions. However, silicon experiences significant volume expansion and contraction during charging and discharging respectively, which causes mechanical stress and results in electrode degradation and battery failure.
It is possible to use lithium metal directly as an anode material. Lithium metal anodes have the highest theoretical capacity and energy density since they are the most lithium dense material. However, the use of metallic lithium anodes presents challenges, such as dendrite formation, which can cause short circuits, safety concerns and reduced cycle life.
Lead–acid batteries feature a Pb-based anode, typically composed of PbO2 on a Pb substrate. NiMH batteries use a hydrogen-absorbing alloy, such as a mixture of nickel (Ni) and metal hydride, as the anode material. The anode absorbs hydrogen ions during charging and releases them during discharge.
Cathode
The cathode is the positive electrode of a cell, associated with reductive chemical reactions.6 Li–ion batteries employ various cathode materials, including lithium cobalt oxide (LCO), lithium iron phosphate (LFP) and lithium nickel manganese cobalt oxide (NMC). These cathode materials can reversibly accept and eject lithium ions into and from out of their crystal structure during charge and discharge cycles.
NiMH batteries typically feature a nickel oxyhydroxide (NiOOH) cathode material. The cathode absorbs hydroxide ions during charging and releases them during discharge. Lithium–air batteries employ a porous carbon-based cathode that interacts with oxygen from the surrounding air, enabling the reversible electrochemical reaction between lithium ions and oxygen during charge and discharge. The reaction on the cathode is electrocatalytic in nature and requires an electrocatalyst.
Current collectors
Current collectors are typically metallic foils or conductive materials that collect and distribute the electrical current generated during battery operation. They are in direct contact with their respective electrodes and are usually made from copper and aluminum due to their high electrical conductivity. Current collectors sometimes act as terminals for the external connection of the individual cells of the battery, allowing electrical current to flow to and from the battery.
How are batteries made and why might you test a battery material?
Before we consider how batteries are made, it is important to note that battery development typically takes a long time and involves progression from the laboratory scale through prototype testing and ultimately to the assembly line and production. The process can be briefly outlined as follows:
- Laboratory research to explore new materials, chemistries and configurations for batteries.
- Proof of concept to demonstrate the viability of promising battery chemistry and materials identified in the lab.
- Prototype development after a successful proof of concept by further refining the battery chemistry and scaling it up.
- Performance evaluation and iterative improvement using extensive testing to assess various performance parameters, such as energy density, power density, cycle life and safety characteristics.
- Pilot production of the optimized prototype design.
- Scale-up and commercial production after successful pilot production.
- Market deployment of commercialized batteries for various applications, such as EVs, portable electronics or grid-scale energy storage.
Significant effort is therefore required before commercialization of a battery chemistry. Building a manufacturing plant can also take several years to commission due to challenges such as the complex value chains, with dozens of suppliers required to source all the materials and components. Most importantly, the final commercial production and manufacturing of the battery also involves several steps from the raw materials to the assembly of battery cells. A typical battery manufacturing process is shown in Figure 5 below.
Modern manufacturing plants use precision manufacturing and high automation to be cost-competitive.15 Rigorous testing is important at all stages to ensure compliance of the final battery with all the necessary specifications. These specifications include but are not limited to safety, cycle life, cost, reusability and sustainability of the manufacturing process. Some areas where battery testing is essential are outlined below.
Figure 5: Schematic of the battery manufacturing process based on Li-ion batteries, detailing the main steps (gray cogs), their corresponding necessary elements (pale blue) and the control measurements and tests (dark blue). Credit: Technology Networks, adapted from Zanotto et al.19
Battery material impurity
Impurities in raw materials can adversely affect battery performance, safety and lifespan. Analytical testing of raw materials helps identify and control impurities to ensure consistent and high-quality battery production. Impurities in electrode materials can hinder electrochemical reactions, reduce capacity and accelerate degradation. Testing of electrode materials helps ensure purity and consistency, leading to optimal battery performance. Almost all the components of the battery are isolated and tested individually.
Battery safety
Heat, flammable and/or toxic gas production are the basic factors that lead to battery failure. Consequently, the safety of a battery system can be improved by firstly avoiding the conditions that lead to heat and gas generation, and secondly, if it does occur, by managing the heat and gas generated to alleviate battery failure. Safety vents and current interruption devices that open in response to pressure increase inside the cell to allow the gases escape are installed in modern batteries to avoid cell rupture. Shut down separators and intelligent battery management systems are also used. All these features are tested for reliability before being introduced into the production line. Significant modifications can also be made to the battery components, such as the cathode, anode or electrolyte, to make them inherently safe.
The failure rate of Li-ion batteries is estimated to be 1 in 40 million if stored and operated within manufacturer-recommended limits.16 However, unpredictable circumstances, such as overcharging, external heating and mechanical abuse, may significantly increase this failure probability. There have been numerous high-profile battery failure accidents, many of which caused significant adverse impacts for the cell manufacturers as well as companies utilizing the specific battery technology within their products. As such, rigorous testing of battery materials, components and related auxiliary systems is performed under harsh conditions to test the “worst case scenario” even if the battery may never experience such conditions under normal use.
Thermal runaway
Thermal runaway is a significant problem associated with batteries, especially Li-ion batteries. It refers to a situation where a battery experiences a self-sustaining and uncontrollable rise in temperature as a result of electrochemical reactions during charge and/or discharge. The heat, when not effectively dissipated, further accelerates other reactions that increase the battery temperature, creating a positive feedback loop. Thermal runaway can potentially result in battery failure, fire or even explosion.
Modern batteries pack a lot of energy. For example, a 55 Ah battery is equivalent to the energy of a hand grenade (150 g of TNT).17 Battery cells or packs are therefore packaged, often with safety features such as protection circuits and thermal management systems. Each of these systems must be tested for precise functionality. Quality control measures, including visual inspection, electrical testing and environmental testing, are implemented to ensure that batteries can control an unexpected temperature rise as well as meet specifications and safety standards set by the various regulatory bodies.
Battery degradation
After battery manufacturing, ongoing monitoring and testing are essential to assess long-term performance, safety and reliability. Material testing is important to identify potential degradation mechanisms, such as electrode material decomposition or electrolyte breakdown. By monitoring these materials, manufacturers can identify improvements in composition or design to enhance battery lifespan and stability. Modern battery management systems have a wide range of functions, including estimation of the state of charge, depth of discharge, state of health and state of function. These together protect the battery from overheating and are useful for data reporting. In effect, each battery produced continues to report critical data to the manufacturer to ensure optimization of processes and identify key components causing battery degradation.
Cost reduction
To stay competitive, battery manufactures need to drive down cost. By testing and understanding material characteristics, manufacturers can optimize battery designs, reduce reliance on expensive or scarce materials and develop more cost-effective production processes. Manufacturers can also identify ways to enhance electrochemical reactions, improve energy storage capacity and extend cycle life. Testing during manufacturing is crucial for meeting regulatory standards and certifications related to safety, environmental impact and performance requirements to avoid the risk of fines or accidents.
Analytical testing in battery manufacturing
Analytical testing is integral to the battery industry to ensure the quality, performance and safety of battery components and products. By employing a range of techniques and analyzing various components, manufacturers can optimize battery performance, identify potential issues and meet the increasing demands for reliable and efficient energy storage systems. These tests are carried out at various stages, including development, production and post-production monitoring using a range of analytical techniques. Manufacturers typically assess the composition, properties and behavior of raw materials, battery slurries, electrodes, electrolytes and other components. An overview of the key aspects of analytical testing is outlined below. This overview is based around Li-ion batteries as one of today’s most common battery types but is not exclusive to Li-ion batteries.
Raw materials analysis:
Raw materials are the starting point of the battery manufacturing process and hence the starting point of analytical testing. The main properties of interest include chemical composition, purity and physical properties of the materials such as lithium, cobalt, nickel, manganese, lead, graphite and various additives. Spectroscopy techniques, such as X-ray fluorescence and atomic absorption, chromatography and elemental analysis help identify impurities, ensure material quality and assess their suitability for battery applications.18 Most of the metals are extracted from their respective ore and also require rigorous analysis during the purification. This is, however, not considered part of the battery manufacturing process.
Battery slurry analysis:
Most battery electrodes consist of electroactive materials coated on the current collector. To coat this active material, the powders are transformed into slurries by mixing with suitable solvents. Battery slurries typically consist of the active materials, binders, conductive additives and solvents. The slurries are evaluated for their viscosity, solid content, particle size distribution and chemical composition. Techniques like rheology measurement help analysts understand the properties of slurries to ensure proper electrode coating and facilitate energy efficient drying of the electrodes to optimize battery performance and reduce cost.19
Electrode analysis:
The electrodes are the heart of the battery where all the electrochemical reactions occur. Testing of the electrodes prior to battery assembly provides insights into their composition, morphology and electrochemical performance. Techniques such as scanning electron microscopy (SEM), energy-dispersive X-ray spectroscopy (EDS), X-ray diffraction (XRD) and Fourier-transform infrared spectroscopy (FTIR) are commonly used. These methods help assess the integrity of electrode structures, identify any defects or impurities and evaluate the effectiveness of active materials. Modern electrochemical testing techniques, such as scanning electrochemical microscopy20,21 and scanning electrochemical cell microscopy,22,23 also enable analysis of single electrodes and electrode materials at the nanoscale for a better understanding of the general electrochemical properties of the electrode.
Electrolyte analysis:
The electrolyte is a critical component of batteries, and its analysis involves examining its stability and ionic conductivity. These properties generally depend on the chemical composition, which is also analyzed. Techniques such as chromatography, mass spectrometry (MS), and nuclear magnetic resonance (NMR) spectroscopy help analyze the electrolyte's constituents, detect impurities and ensure proper ionic mobility. Electrochemical impedance spectroscopy (EIS) is another technique used to assess the performance of electrolytes in terms of resistance and ion diffusion.
Battery performance testing:
Analytical testing also involves evaluating battery performance parameters such as capacity, energy density, cycle life and safety characteristics. Techniques like cyclic voltammetry, galvanostatic charge-discharge testing, differential scanning calorimetry (DSC) and thermal stability analysis are usually employed. These tests assess the battery's ability to store and deliver energy efficiently, monitor its degradation over time and ensure compliance with safety standards.24
Post-production monitoring:
After battery production, ongoing analytical testing is essential for post-production monitoring and quality control. This includes periodic sampling and testing of battery batches to verify consistency and compliance with specifications. Techniques used during this phase may involve the same methods mentioned earlier, with a focus on performance verification, safety assessment and batch-to-batch consistency.
Batteries research and green batteries
The increasing demand for energy storage in various sectors, including EVs and renewable energy systems, makes battery development a promising technological field.25 Automakers are striving to increase EV driving ranges, reduce charging times and enhance overall vehicle performance. Battery technology will continue to evolve, aiming for higher energy densities, longer cycle life, faster charging capabilities, improved thermal management and safety. Researchers will keep exploring novel electrode materials, such as silicon-based anodes, solid-state electrolytes and advanced cathode chemistries (e.g., nickel-rich formulations) to enhance overall performance.
With growing emphasis on developing environmentally friendly and sustainable batteries, a reduction in the reliance on critical raw materials like cobalt and nickel, which are often associated with social and environmental concerns, is expected. Alternative chemistries using more abundant and environmentally friendly materials like sodium–ion, zinc–ion, and lithium–sulfur batteries may become popular.
Safety concerns currently associated with Li-ion batteries continue to drive the development of solid-state batteries offering benefits like higher energy density, improved safety and wider operating temperature ranges.26 While still in the research and development phase, solid-state batteries have the potential to revolutionize energy storage applications, especially in EVs. The battery industry will play a crucial role in meeting these demands through continuous innovation and scaling up production capacities. Grid-scale energy storage systems, utilizing large-scale batteries, will be necessary for stabilizing electricity grids, managing peak demand and storing excess renewable energy from sources like solar and wind power.
The battery industry will need to develop cost-effective and efficient solutions to meet the growing demands of grid-scale energy storage. In particular, as the volume of batteries in use increases, the importance of battery recycling and second-life applications is being emphasized. Establishing robust recycling infrastructure and processes will be essential to recover valuable materials and minimize environmental impact. For example, retired EV batteries can be either recycled or repurposed for stationary energy storage, extending their useful life and reducing waste. As with many industries, future battery research and manufacturing will likely experience significant digitalization15 and benefit from computer assistance such as machine learning27 and artificial intelligence.28
1. Sarma DD, Shukla AK. Building better batteries: A travel back in time. ACS Energy Lett. 2018;3(11):2841-2845. doi:10.1021/acsenergylett.8b01966
2. Goodenough JB. How we made the li-ion rechargeable battery. Nat Electron. 2018;1(3):204. doi:10.1038/s41928-018-0048-6
3. Goodenough JB, Park K-S. The li-ion rechargeable battery: A perspective. J Am Chem Soc. 2013;135(4):1167-1176. doi:10.1021/ja3091438
4. Dutta A, Mitra S, Basak M, Banerjee T. A comprehensive review on batteries and supercapacitors: Development and challenges since their inception. Energy Storage. 2023;5(1). doi:10.1002/est2.339
5. Lankey RL, McMichael FC. Life-cycle methods for comparing primary and rechargeable batteries. Environ Sci Technol. 2000;34(11):2299-2304. doi:10.1021/es990526n
6. Winter M, Brodd RJ. What are batteries, fuel cells, and supercapacitors? Chem Rev. 2004;104(10):4245-4269. doi:10.1021/cr020730k
7. Smith MJ, Gray FM. Batteries, from cradle to grave. J Chem Educ. 2010;87(2):162-167. doi:10.1021/ed800053u
8. Arora P, Zhang ZJ. Battery separators. Chem Rev. 2004;104(10):4419-4462. doi:10.1021/cr020738u
9. Volta A. On the electricity excited by the mere contact of conducting substances of different kinds. In a letter from Mr. Alexander Volta, f. R. S. Professor of natural philosophy in the University of Pavia, to the rt. Hon. Sir Joseph Banks, bart. K. B. P. R. S. Philosophical Transactions of the Royal Society of London. 1800;90. Accessed June 7, 2023. http://www.jstor.org/stable/107060
10. Zhang H, Liu X, Li H, Hasa I, Passerini S. Challenges and strategies for high-energy aqueous electrolyte rechargeable batteries. Angew Chem Int Ed. 2021;60(2):598-616. doi:10.1002/anie.202004433
11. Liang Y, Yao Y. Designing modern aqueous batteries. Nat Rev Mater. 2023;8(2):109-122. doi:10.1038/s41578-022-00511-3
12. Frith JT, Lacey MJ, Ulissi U. A non-academic perspective on the future of lithium-based batteries. Nat Commun. 2023;14(1):420. doi:10.1038/s41467-023-35933-2
13. Wang F, Borodin O, Ding MS, et al. Hybrid aqueous/non-aqueous electrolyte for safe and high-energy li-ion batteries. Joule. 2018;2(5):927-937. doi:10.1016/j.joule.2018.02.011
14. Famprikis T, Canepa P, Dawson JA, Islam MS, Masquelier C. Fundamentals of inorganic solid-state electrolytes for batteries. Nat Mater. 2019;18(12):1278-1291. doi:10.1038/s41563-019-0431-3
15. Ayerbe E, Berecibar M, Clark S, Franco AA, Ruhland J. Digitalization of battery manufacturing: Current status, challenges, and opportunities. Adv Energy Mater. 2022;12(17):2102696. doi:10.1002/aenm.202102696
16. Wang Q, Mao B, Stoliarov SI, Sun J. A review of lithium ion battery failure mechanisms and fire prevention strategies. Prog Energy Combust Sci. 2019;73:95-131. doi:10.1016/j.pecs.2019.03.002
17. Dubarry M, Baure G. Perspective on commercial li-ion battery testing, best practices for simple and effective protocols. Electronics. 2020;9(1):152. doi:10.3390/electronics9010152
18. Konarov A, Voronina N, Jo JH, Bakenov Z, Sun Y-K, Myung S-T. Present and future perspective on electrode materials for rechargeable zinc-ion batteries. ACS Energy Lett. 2018;3(10):2620-2640. doi:10.1021/acsenergylett.8b01552
19. Zanotto FM, Dominguez DZ, Ayerbe E, et al. Data specifications for battery manufacturing digitalization: Current status, challenges, and opportunities. Batter Supercaps. 2022;5(9). doi:10.1002/batt.202200224
20. Santos CS, Botz A, Bandarenka AS, Ventosa E, Schuhmann W. Correlative electrochemical microscopy for the elucidation of the local ionic and electronic properties of the solid electrolyte interphase in li-ion batteries. Angew Chem Int Ed. 2022;61(26):e202202744. doi:10.1002/anie.202202744
21. Strange LE, Li X, Wornyo E, Ashaduzzaman M, Pan S. Scanning electrochemical microscopy for chemical imaging and understanding redox activities of battery materials. Chem Biomed Imaging. 2023;1(2):110-120. doi:10.1021/cbmi.3c00014
22. Tetteh EB, Valavanis D, Daviddi E, et al. Fast li-ion storage and dynamics in tio2 nanoparticle clusters probed by smart scanning electrochemical cell microscopy. Angew Chem Int Ed. 2023;62(9):e202214493. doi:10.1002/anie.202214493
23. Martín-Yerga D, Milan DC, Xu X, et al. Dynamics of solid-electrolyte interphase formation on silicon electrodes revealed by combinatorial electrochemical screening. Angew Chem Int Ed. 2022;61(34):e202207184. doi:10.1002/anie.202207184
24. Chen R, Li Q, Yu X, Chen L, Li H. Approaching practically accessible solid-state batteries: Stability issues related to solid electrolytes and interfaces. Chem Rev. 2020;120(14):6820-6877. doi:10.1021/acs.chemrev.9b00268
25. Silva J, Távora G, Mendonça S. Reconfiguring the energy storage landscape. Foresight and STI Governance. 2023;17(1):34-50. doi:10.17323/2500-2597.2023.1.34.50
26. Janek J, Zeier WG. Challenges in speeding up solid-state battery development. Nat Energy. 2023;8(3):230-240. doi:10.1038/s41560-023-01208-9
27. Gao T, Lu W. Machine learning toward advanced energy storage devices and systems. iScience. 2021;24(1):101936. doi:10.1016/j.isci.2020.101936
28. Lombardo T, Duquesnoy M, El-Bouysidy H, et al. Artificial intelligence applied to battery research: Hype or reality? Chem Rev. 2022;122(12):10899-10969. doi:10.1021/acs.chemrev.1c00108