Synthetic Biology: Engineering Meets Biology
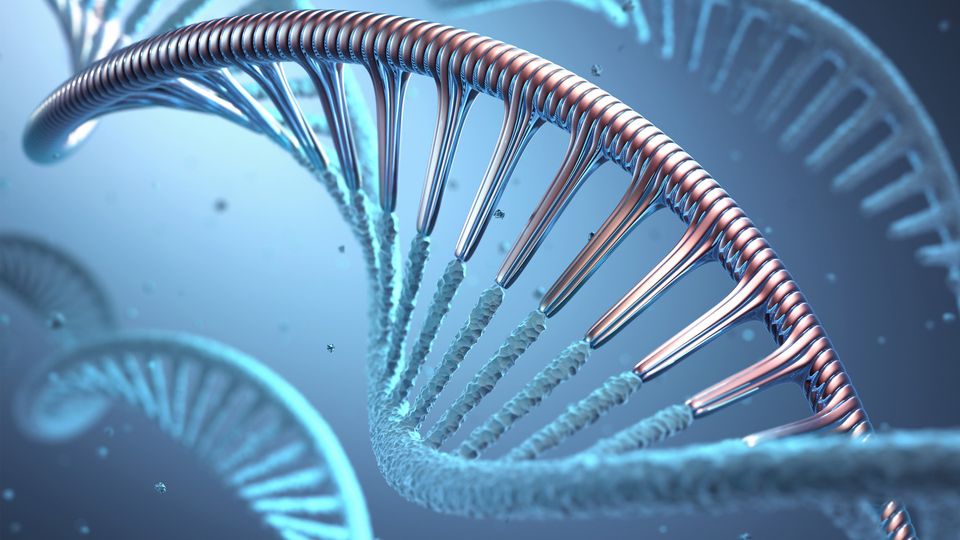
Complete the form below to unlock access to ALL audio articles.
For a long time, the term synthetic biology evoked, for many, imagery of futuristic scenes of “fabricated” organisms from science fiction movies, instead of an established scientific discipline as it stands today. The concept of synthetic biology emerged from early studies in E. coli of an “operon”, a collection of genes within a stretch of functional DNA that have regulatory functions that dictate biological activity. If an operon could be rationally manipulated, novel regulatory and biological activity could be designed into organisms. These early concepts morphed into reality with the advent of molecular biology, and, for the past few decades, synthetic biology has evolved and advanced rapidly, growing in scope and application. The field is truly transdisciplinary; the synthetic biology literature is peppered with both biology and engineering concepts. Terms such as microorganism, transcription and translation in publications occur alongside concepts describing toggle switches, oscillators and logic and autoregulatory circuits.
As a heterogenous field encompassing a breadth of disciplines, including biology, chemistry, engineering and computational and systems science, the term synthetic biology may appear nebulous. However, in essence, it can be regarded as the rational and intentional design of cellular characteristics using molecular biology. Cellular behavior or outcomes are dictated by integrating designed “circuits” into cells, functional DNAs with regulatory activity that respond to external cues to generate an intended outcome or behavior. Earlier applications revolved around E. coli bacteria and S. cerevisiae yeast, whose regulatory gene circuits were more easily cataloged. However, mammalian cells are now also routinely engineered. Additionally, studies have moved past purely transcriptional control to encompass post-transcriptional and translational control through engineered RNAs.
To tweak or not to tweak?
Engineering efforts in synthetic biology can take a couple of directions. “The more straightforward approach, perhaps, is to make use of an existing circuit within the cellular machinery and modify or redirect it, repurposing it for your needs,” explained Daniel Bojar, professor at the Wallenberg Centre for Molecular and Translational Medicine at the University of Gothenburg. “Because an existing circuit operates in the context of its cellular origin, and is already well-integrated into the cellular machinery,
an engineered receptor can potently regulate that circuit, effectively modulating cellular output behavior,” Bojar elaborated. “On the other hand, this engineered pre-existing circuit may function differently when integrated into another cellular environment, leading to more variable and unpredictable behavior across cell types, limiting generalizability and modularity.”
Since an overarching goal of synthetic biology is to create modular circuits that can be assembled following simple rules to generate an intended outcome, this lack of generalizability can be a hurdle. Another approach for synthetic biologists is to build circuits de novo, i.e., from scratch. However, this avenue faces its own set of obstacles, not least of which are the difficulties inherent to the design of circuits meant to integrate into highly complex cells. Or, alternatively, de novo design might generate multiple modular circuits, but then faces the challenge of assembling the circuits in a manner that will lead to the desired output. “Ultimately, I think, the design process is a careful balancing act. We want circuits to be modular, transferable and generalizable, but need to balance modularity with capitalizing on existing biological machinery,” Bojar explained.
Up against nature
Another important challenge facing synthetic biologists is the issue of robustness. “The systems we design can be very fragile for numerous reasons. For instance, the synthetic gene circuits and metabolic pathways we create generally reduce the fitness of cells, rendering them susceptible to environmental pressures,” explained Matthew R. Bennett, professor in the Department of BioSciences at Rice University. “Consequently, it is common to see mutations emerge in our circuits as microorganisms evolve under selective pressure from environmental stress. While these mutations may increase cellular fitness and help the microorganism adapt, they generally reduce circuit function, and, thereby, disrupt the original design.”
Environmental factors are also an issue in microbial consortia, a group or colony of microorganisms designed to respond to external cues in concert, a particular area of Bennett’s expertise. “Many of our synthetic designs assume a uniform environment; however, that is usually not the reality in nature, which is highly complex and heterogeneous, so we need to build circuits within consortia that are resistant to fluctuations in the environment,” Bennett elaborated. “Additionally, we still do not fully understand how environmental factors can alter the interactions of a synthetic gene circuit with cellular biochemistry. These issues are becoming increasingly problematic as we envision synthetic systems that would be employed in complex environments for long periods of time.”
Say hello to your neighbor! Consortia of communicating cells
The challenge of navigating environmental factors is one Bennett faces in his research designing synthetic circuits of microbial consortia, which must communicate intercellularly across members. “We achieve intercellular communication by leveraging quorum sensing molecules, which microbes use naturally to sense neighbors. These molecules, which are N-acyl homoserine lactones, modulate gene expression of operons that regulate cell division and hence population size,” Bennett explained.
Bennett and his team demonstrated how a synthetic oscillating circuit, i.e., fluctuating output between two states, could be built into a microbial consortium. “We employed two E. coli strains, one called the ‘activator’ strain, producing C4-homoserine lactone that enhances gene expression, and one called the ‘repressor’ strain, producing 3-OHC14-homoserine lactone that represses gene expression,” Bennett described. “When cultured together, the two strains generated a consortium that oscillated on and off in relative gene expression.” To visualize the oscillations, gene expression was tied to cyan fluorescent protein expression in the activator and yellow fluorescent protein expression in the repressor. The microbial consortium, grown within a chamber of a microfluidic device, could be imaged and showed a cyan band of activators neighboring a yellow band of repressors, lighting up and turning off in cycles.
Bennett also tested the parameters of the synthetic oscillator. “Of course, both strains had to be present for the oscillator to function. However, we found we could modulate the period of oscillations as well as the shape of each wave by varying the circuit topology (i.e., the number of positive and feedback loops). Importantly, the ratio of activator to repressor E. coli did not affect oscillations, meaning the circuit was not dependent on population dynamics.”
Consortia circuits are more robust and efficient than isogenic systems, i.e., circuits built using a single strain based solely on intracellular responses, because they distribute the metabolic load across two or more strains. However, consortia are hard to control and must be carefully organized spatially and temporally by optimizing communication. This is limited by the distance quorum sensing molecules can traverse, only on the order of a few microns to centimeters, constraining consortia size. Moreover, complex and heterogenous environments further hinder the dispersion of quorum sensing molecules. To address these challenges, Bennett examined how gene expression could be temporally coordinated long-range to facilitate communication across synthetic microbial consortia. “Using our dual system of activator and repressor, we built our synthetic circuits within microfluidic devices with larger chambers, so the consortia had more space to grow. We found that a certain positive feedback loop within the circuit, which augmented and transmitted intercellular signals, was critical for long-range coordination,” Bennett concluded, highlighting this important step towards realizing larger and more robust consortia.
Good morning! Caffeine switches gene expression on
A useful tool in the synthetic biologist’s toolbox is a circuit responsive to external cues for “on-demand” expression of a certain gene, which could have many important potential applications. In a similar vein, Bojar developed a caffeine-inducible gene switch during his time in the Fussenegger lab. “Our goal was to make the circuit responsive to caffeine levels that would be present in circulation after drinking a cup of coffee, so in the low micromolar range. We went through a few iterations, but our final design was based on an antibody with a caffeine-binding domain and a signal transduction domain from interleukin-6 receptor subunit beta (IL6-RB). Caffeine binding would induce antibody dimerization, which would also bring two IL6-RB signaling domains together,” Bojar outlined. “IL6-RB dimerization would emit and amplify a signaling cascade culminating in a transcription factor ‘receiver’, which contained a transcription-activating domain on a circuit switch. This would switch the circuit on, which would start expressing your gene of interest in response to the original caffeine input.”
Bojar first tested the caffeine-inducible switch by expressing a reporter gene in a stem cell-derived cell line. “We found the switch had a broad dynamic range, and dose-dependently responded to caffeine at varying concentrations, from 10 nanomolar to 100 micromolar. It responded quite quickly, within hours, and the signal intensity depended on caffeine levels,” Bojar elaborated on the switch’s characteristics. “Importantly, for potential biomedical applications, the switch was reversible and could be turned off by washing caffeine out of culture.”
Bojar next assessed if the caffeine-inducible switch could be used to control the expression of glucagon-like peptide 1 (GLP-1), a glucose-regulating peptide hormone. GLP-1 receptor agonists are part of the repertoire of pharmacological agents for treating type 2 diabetes. “An inducible GLP-1-expressing circuit could potentially regulate hyperglycemia in mice with obesity and type 2 diabetes,” Bojar explained. A cell line transfected with the caffeine-inducible GLP-1 circuit was microencapsulated and administered to two animal models. Upon administering coffee to prediabetic mice with diet-induced obesity, the caffeine-inducible GLP-1-expressing cells lowered fasting glucose, improved glucose tolerance and reduced weight gain in tandem with increased levels of circulating GLP-1 and insulin. “We also saw beneficial effects in the type 2 diabetes model of leptin receptor-deficient (db/db) mice. Coffee consumption following administration of caffeine-inducible GLP-1-expressing cells also improved glucose tolerance and circulating insulin and GLP-1 levels,” Bojar concluded of the study, which demonstrated a potential biomedical utilization of synthetic biology.
Manyfold potential applications
There are many potential applications of synthetic biology, such as understanding basic biology, technological applications (e.g., biosensors and CRISPR-based computation) and biomedical and pharmaceutical applications. During his time in the Fussenegger lab, Bojar worked on numerous biomedical applications, including insulin-secreting cells, circuits inducible by metabolically inert L-glucose and designer exosomes (dubbed EXOtic devices) for treating a mouse model of Parkinson’s disease. Bojar envisions further medical uses, such as CAR-T cells or making organs compatible for transplantation.
However, he is also very enthusiastic about basic research. “With all the exciting emerging biomedical applications, basic research has been a bit neglected, although I think it is an equally prominent area,” Bojar concluded. “Given the powerful modulating tools that synthetic biology provides, we stand to gain both mechanistic and causal insights, for instance using live-sensing metabolites or by disentangling signaling pathways via specific reporters downstream. We have at our disposal some really powerful genetic engineering tools. Although much progress has been made, we have only just scratched the surface and I think many exciting discoveries lie ahead.”
Bennett echoed this sentiment. “There are so many exciting applications. In the short-term these include medical applications, such as CRISPR-based gene editing, bacterial therapeutics and biomaterials (i.e., cells embedded in materials to provide novel functions). In the long-term, I hate to even speculate,” Bennett concluded. “As our ability to engineer biology increases, the number of possibilities explodes. I believe that the killer application for synthetic biology has not been envisioned yet. Just as the first computer scientists probably never could have predicted smartphones, streaming devices and AI, I don’t think we can predict what our work will eventually become. But it will be amazing, of that I have no doubt.”